Interspecific differences and ecological correlations of ultraviolet radiation tolerance in low- and high-altitude fishes
- 1Integrative Science Center of Germplasm Creation in Western China (CHONGQING) Science City & Southwest University, Chongqing, China
- 2Key Laboratory of Freshwater Fish Reproduction and Development (Ministry of Education), Key Laboratory of Aquatic Science of Chongqing, Southwest University, Chongqing, China
- 3School of Life Sciences, Southwest University, Chongqing, China
- 4QuJing NO.1 Middle School Qing Yuan Campus, QuJing, China
- 5College of Fisheries, Southwest University, Chongqing, China
Ultraviolet radiation (UVR) is a ubiquitous environmental factor and with complex and diverse effects on organisms, and the UVR tolerance of species varies due to selection, adaptation and evolution. This study aimed to improve our understanding of the interspecific differences in UVR tolerance of fishes. First, we compared skin histology between high-altitude fishes and their low-altitude relatives. Second, we assessed the skin histological changes of 22 fish species after UVR exposure and quantified their damage levels. Finally, the factors affecting the difference in UVR tolerance among fishes were studied. Thin or absent scales was a common characteristic of high-altitude fishes in different taxonomic groups, but other skin structure parameters (the thickness of the dermis and epidermis, the size and number of mucous and club cells) did not show convergence. After UVR exposure, the size and number of mucous cells and club cells in the experimental fishes were generally reduced, but the changes in epidermal and dermal thickness varied among species. The change rata of melanin area was strong negatively correlated with the level of damage, indicating that increased melanin was helpful to reduce UVR damage. On the whole, the skin histological changes of high-altitude fishes after UVR exposure did not differ from those of low-altitude fishes. However, the epidermis and dermis of Gymnocypris eckloni and Oncorhynchus mykiss, which had the highest UVR tolerance, were nonpathologically thickened, indicating that they may contribute to UVR tolerance. There were significant interspecific differences in UVR tolerance among the experimental fishes, with high-altitude and cold water fishes showing stronger UVR tolerance. Interestingly, some low-altitude cold water fish (e.g., O. mykiss) also showed high UVR tolerance, suggesting that besides high-altitude adaptation, low-temperature adaptation also may play an important role in organismal adaptation to UVR.
Introduction
Ultraviolet radiation (UVR) is a ubiquitous environmental factor, and its intensity changes with time and space; it is stronger at low latitudes and high elevations and in summer than in winter (Holzle and Honigsmann, 2005; Beckmann et al., 2014). The complex and diverse effects of UVR on the physiology, distribution and population dynamics of numerous organisms are being increasingly recognized (Paul and Gwynn-Jones, 2003; Kessler et al., 2008; Leech et al., 2009; Gautam et al., 2010; Ballare et al., 2011; Beckmann et al., 2014). Differences in UVR tolerance among fish have long been recognized (Fabacher and Little, 1995; Blazer et al., 1997; Fukunishi et al., 2006), but only a few species have been compared, and the associated studies failed to provide mechanistic explanations or employ ecological correlation analyses.
High natural UVR exposure has deleterious consequences for aquaculture fish species inhabiting shallow waters, such as tanks, ponds, and cages in offshore areas (Bullock, 1982; Zagarese and Williamson, 2001; Williamson et al., 2019; Alves et al., 2020). Skin damage is the most common harmful effect of UVR exposure in fish. Signs of sunburn, hyperpigmentation, disruption of the mucous layer, epidermal edema, necrosis, and sloughing of the epidermis are some of the consequences of UVR overexposure on fish skin (Bullock, 1988; Ewing et al., 1999; Manek et al., 2012; Abedi et al., 2015). The epidermis of fish mainly consists of mucous cells (goblet cells), club cells (alarm substance cells) and several layers of flattened stratified epithelial cells. Mucous cells are sensitive to UVR exposure,with their number and size tending to decrease after exposure (Blazer et al., 1997; McFadzen et al., 2000; Sucre et al., 2012; Abedi et al., 2015). Club cells may be important in the protection against UVR damage, e.g., some fishes had significantly more club cells on their dorsal surface than their flank (Hugie, 1990; Chivers et al., 2007), and some fishes had significantly more and larger club cells after UVR exposure (Blazer et al., 1997). However, in other studies, some fish had fewer and smaller club cells under UVR exposure (Manek et al., 2012; Manek et al., 2014). These contradictory results indicate that the responses of club cells to UVR exposure differ among species, which may also be due to differences in experimental conditions. However, multispecies studies carried out under the same experimental conditions are lacking.
Among all orogenic belts on Earth, the Tibetan Plateau is known as the “roof of the world” due to its vast area and high average elevation (>4000 m above sea level). The uplift of the plateau has significantly affected the climate, caused geoenvironmental changes in Asia and created a unique highland habitat with extreme coldness, high UVR, low oxygen, and in some places, severe aridity, which has attracted extensive attention from biologists and paleontologists (Qu et al., 2013; Wang et al., 2015; Yuan et al., 2020; Liu et al., 2021a; Mao et al., 2021; Yang et al., 2021a). However, numerous fish are adapted to such extreme conditions, including Schizothoracinae (family: Cyprinidae), Sisoridae (superfamily: Sisoroidea; order: Siluriformes), and Triplophysa (family: Nemacheilidae; order: Cypriniformes). High-altitude lakes and rivers have clear water with low UVR attenuation (Bullock, 1988; Liu et al., 2021b). Therefore, high-altitude fish experience greater UVR pressure. Skin is the first defense layer against UVR. Therefore, the skin structure of high-altitude fish may undergo adaptive evolution. However, previous studies on the adaptation of fish to high-altitude environments mainly focused on extreme coldness and low oxygen and employed genetic analysis (Xiao et al., 2020; Yuan et al., 2020; Yang et al., 2021b), and little attention was given to UVR adaptation. In addition, current research on fish high-altitude adaptation mainly involves the genetic analysis approach and lacks experiments and phenotypic comparisons.
Investigating differences in UVR tolerance and associated phenotypes is the first step in gaining a deeper understanding of the mechanisms involved. In this study, we compared UVR tolerance and skin histology before and after irradiation among 22 fish species and asked which factors contributed to their differences in tolerance. Specifically, we aimed to answer three main scientific questions: (1) What are the differences in the skin structure of high-altitude species relative to their low-altitude relatives? (2) What are these differences in tolerant vs. less tolerant species? What are they in less tolerant species? (3) Which factors cause differences in UVR tolerance?
Materials and methods
Experimental animals
First, the differences in skin histology of high-altitude fishes (Schizothoracinae (family: Cyprinidae), Sisoridae (superfamily: Sisoroidea; order: Siluriformes), and Triplophysa) and their low-altitude relatives were assessed (Table S1). Second, the skin structure change among 22 species after UVR exposure were determined (Table S3). Sources of the experimental fish include (1) the artificial breeding of offspring of wild species. The species were collected by fishing net or minnow traps in the wild, and bred to sexual maturity in the artificial culture environment, and the offspring were obtained by artificial insemination. (2) Wild species. The species were collected by fishing nets, minnow traps or fishing rods in the wild. (3) Aquaculture species. Before the start of the experiment, all species were temporarily kept in an indoor recirculating aquaculture system at a suitable temperature and fed commercial feedstuff (Citico, T3, 40% crude protein). We referred to previous studies (Ding, 1994; Chen, 1998; Guo et al., 2021) and our laboratory’s long-term field investigation experience to classify the habitat water temperature and altitude distribution of each experimental fish (this information such as classification, length and origin of species can be found in Tables S1 and S3). The experimental fishes were divided into high-altitude (average altitude > 1000 m) and low-altitude (average altitude< 1000 m) fishes, and cold water (temperature range: 0-25°C) and eurythermal fishes (temperature range: 0-32°C) (Table S3).
Histology
In the comparison of skin histology between high-altitude species and low-altitude species, to reduce the error caused by differences in the culture environment, all experimental fish were cultured in aerated tap water for 3 days before skin tissue collection, and since both cold water fish and eurythermal fish were examined, an intermediate culture temperature (20°C) was set. Then, the fish were euthanized with an overdose of MS-222 (tricaine methane sulfonate, 500 mg/L), and their body width was measured. Next, lateral skin tissue (above the lateral line and anterior to the dorsal fin, with a length of 0.5-1.0 mm and a width of 0.3-0.6 mm, Figure 1A) was collected, fixed in 4% paraformaldehyde for approximately 12 hours at 4°C and then transferred to a 70% alcohol solution for long-term preservation. Although the tissues included scales, the scales of juvenile fish are not well developed, so we did not decalcify the tissues to soften the scales. Then, the skin tissues were placed in plastic cassettes and processed (gradual dehydration in 70–100% alcohol, clearing in xylene, and embedding in paraffin wax). Traditional paraffin sections (6 μm) were obtained using a microtome (Leica 2245, Leica, Germany), and 30-60 approximately consecutive sections per sample were retained, they were attached to 3 slides, 10-20 sections per slide. These sections were stained with an AB-PAS staining kit (DG1014-6×100ML, Dingguo). All sections were observed using a microscope (Nikon SMZ25, Japan). A complete and representative section was selected for each sample, and three random images of this section were photographed (100×). Each image was measured using ImageJ software (National Institute of Health, Bethesda, MD). We sequentially measured the thickness of the epidermis (outermost layer), scales and dermis in each image(with a representative area of intermediate thickness selected). Next, 10 mucous cells and 10 club cells were randomly selected in each image, and their mean cross-sectional area was calculated. Then, we measured the length of the skin tissue and counted the number of all mucous cells and club cells in each image to calculate their number per unit length in skin cross section (n/mm) (Figure 1B). Finally, we calculated indicators for each image, including the relative epidermis thickness (RET), relative scale thickness (RST), relative dermal thickness (RDT), mean area of mucus cells (MAMC), mean area of club cells (MACC), relative total area of mucous cells (RTAMC), relative total area of club cells (RTACC), using the following formulas:
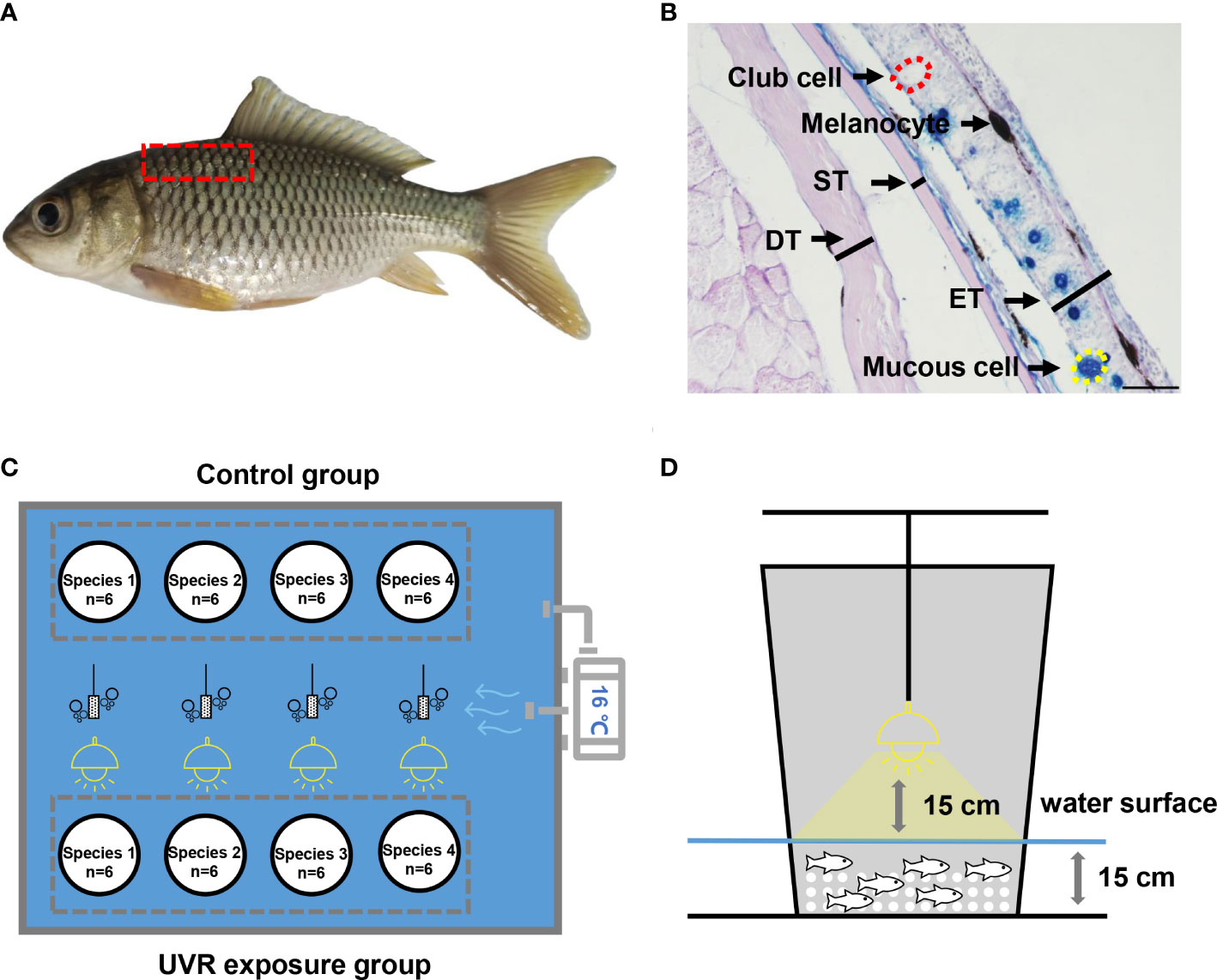
Figure 1 Schematic diagram of experimental methods. (A) Skin tissue sampling area. (B) Histological measurement. ET, epidermal thickness; ST, scale thickness; DT, dermal thickness. The scale is 50 μm. (C) Plane diagram of the UVR exposure experiment. (D) Sectional diagram of UVR exposure experiment.
Where ET represents the epidermis thickness, ST represents the scale thickness, DT represents the dermal thickness, BW represents the body width. These indicators are divided by body width to eliminate the effect of fish size. MAMC represents the mean area of mucous cells, NMC represents the number of mucous cells, MACC represents the mean area of club cells, and NCC represents the number of club cells. These indicators are divided by body width squared to eliminate the effect of fish size (considering that both RTAMC and RTACC are area data divided by body width squared to eliminate units). The above indicators are multiplied by 104 or 105, so that most of the final values are between 10~100. The same methods and indicators are used in our previous study (Gu et al., 2022).
UVR exposure
In an indoor pond (2.7 m × 3.4 m, in a transparent greenhouse), eight 65 L white breeding buckets (with a bottom diameter of 34 cm and a large number of 6-8 mm diameter holes punched in the wall of the barrel to maintain water exchange) were divided into 4 experimental groups and 4 control groups, allowing simultaneous experiments on 4 species. The experimental groups were on one side, and the control groups were on the other side. There were 4 equally spaced oxygenating heads in the pool, which provided continuous oxygenation for 24 hours. The buckets in the experimental group were placed below a 275 W full-spectrum solar lamp (Sparkzoo, China). In contrast, the buckets in the control group were covered with a transparent acrylic sheet, which resulted in nearly undetectable UVR. We set the experimental UVA and UVB daily dose based on the natural doses of solar UVA and UVB radiation in the Lhasa area of the Qinghai-Tibet Plateau in summer; the average is approximately 1.50×103 kJ/m2/d (UVB/UVA ≈ 0.07~0.10) (Peng et al., 2015; Weise et al., 2018; Zhao et al., 2018; Norsang et al., 2019). These sun lamps were suspended 15 cm above the water surface, and the light reached the entire water surface. At this height, the central doseof solar UVA and UVB radiation was approximately 1.08×102 kJ/m2/h (UVB/UVA ≈ 0.09) as measured by a multiprobe ultraviolet irradiator (LS125, Linshang, China). The water depth (local groundwater) in the bucket was kept at approximately 15 cm to maintain activity space for the experimental fish and prevent excessive UVR loss. The water temperature was controlled at 16 ± 1°C (close to the natural water temperature at the beginning of the experiment) by a temperature controller (CW-5000A, Resun, China), and the water was evenly distributed by a water pump (Figures 1C, D).
The experimental and control groups of each species included 6 individuals each. The experimental groups received irradiation by a sun lamp every day for 7 days from 7:00 to 19:00 (Date: March-April; Location: Chongqing, China; Altitude: 250 m), and the UVA and UVB daily dose was approximately 1.30×103 kJ/m2/d. The dorsal skin tissue of all fish was collected and fixed on the evening of the seventh day, and then paraffin sections, AB-PAS staining and quantification were performed. In addition to the above indicators, we additionally measured the total area of melanocytes (TAM) of all fish per unit length of skin (1 mm) using the color range selection function in Adobe Photoshop 13.0 (Adobe, San Jose, CA, USA). Similarly, they were divided by body width squared to eliminate the effect of fish size, and the relative total area of melanocytes (RTAM) was calculated using the following formulas:
In addition, the change rate of above all indicators was calculated using the following formulas:
Where MC represents the mean of control group; ME represents the mean of experimental group.
At the end of the 7-day exposure experiment, no fish in the control group had died, but some fish in the experimental group had died and thus were not subjected to tissue sectioning.
Damage levels
The damage caused by UVR exposure was classified into 0-6 levels based on previous studies (Bullock, 1988) and slightly modified according to the experimental conditions (Figure S1).
Level 0: Histologically, the epidermis in the exposed group changed, but there was no obvious damage.
Level 1: Histologically, the epidermis in the exposed group did not slough off, but there were obvious changes in cell size, such as smaller mucous cells and club cells.
Level 2: Histologically, the epidermis in the exposed group did not slough off, but there were obvious changes in cell structure, such as irregular structures of mucous cells and club cells.
Level 3: Histologically, initial sloughing of the outer epidermal layer occurred.
Level 4: Histologically, complete sloughing of the epidermal layer occurred.
Level 5: Death occurred after UVR exposure. In addition, the epidermis showed obvious damage, such as shedding and necrosis.
Some species may also die from internal physiological factors after UVR exposure, so the experimental individuals that died but had no obvious skin damage were excluded from subsequent analyses.
Phylogenetic analysis
The 13 protein-coding gene sequences of complete mitochondrial genome of all experimental fishes were downloaded, and the DNA sequences were then aligned using the MAFFT Online Service (Katoh et al., 2019). All sequences were obtained from GenBank (https://www.ncbi.nlm.nih.gov/nuccore/), and GenBank accession numbers of each species are provided in Tables S1 and S3. In PhyloSuite v1.2.1 (Zhang et al., 2020), the 13 protein-coding genes were partitioned by the first, second, and third codons in. Finally, a maximum likelihood phylogenetic tree was constructed using the default setting of IQ-TREE with default models (Trifinopoulos et al., 2016).
Statistical analyses
All data were expressed as the mean ± standard deviation (SD) and subjected to oneway ANOVA after normality and homogeneity were checked using IBM SPSS Statistics (version 21.0, Armonk, New York, United States). When ANOVA identified that overall differences were significant (P< 0.05), Tukey’s multiple range test was used to test the difference between treatments. In this study, 0.0<│r│≤0.25 indicated no correlation, 0.25<│r│≤0.5 indicated a weak correlation, 0.5<│r│≤0.75 indicated a moderate correlation, and 0.75<│r│≤1.0 indicated a strong correlation (Bae et al., 2006). To determine the impact of phylogeny on the relationships between the relative scale thickness and UVR damage, we performed phylogenetic independent contrasts (PIC) (Felsenstein, 1985) to determine whether strong relationships existed between them without the influence of phylogeny. PIC analysis was completed by the pic function of the APE package (Paradis et al., 2004) in R software. All graphs were generated by Origin software (Electronic Arts Inc, California, USA) and Excle 2022 (Microsoft, USA).
Results
Comparison of skin histology between high-altitude and low-altitude fishes
In Cyprinidae, both Schizothorax and Gymnocypris belong to the Schizothoracinae (in terms of morphology), but they are not monophyletic groups according to molecular analysis (Wang et al., 2013; Yang et al., 2015), which means that the high-altitude adaptation of cyprinids occurred independently at least twice. The RST of the three high-altitude fishes (Schizothorax davidi, Percocypris pingi and Gymnocypris eckloni) was significantly lower than that of most low-altitude fishes. However, there were no uniform differences in other skin histological indicators between high-altitude fishes and low-altitude fishes. Interestingly, Gymnocypris eckloni had almost no club cells in the epidermis (Figure 2 and Table S2).
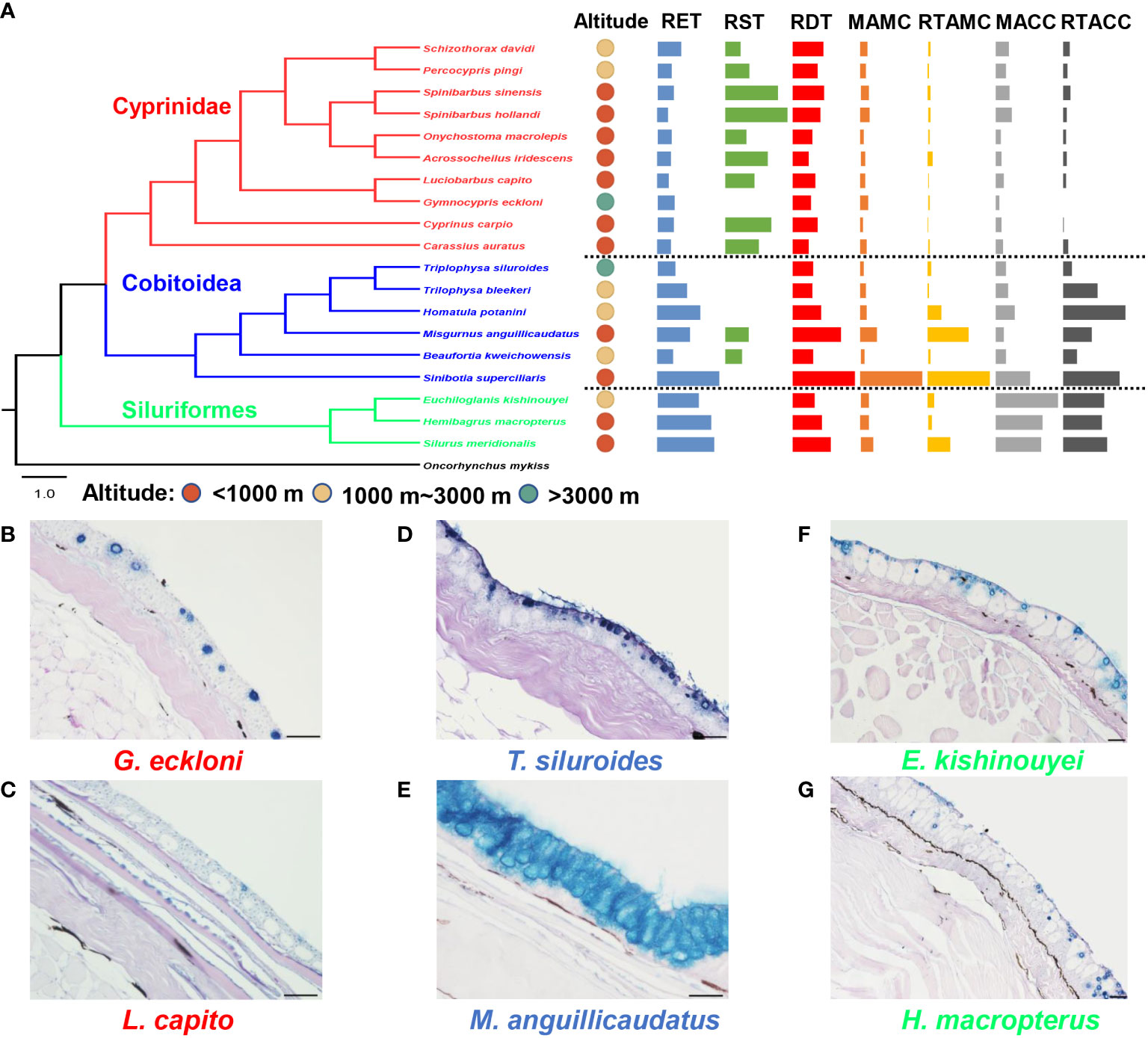
Figure 2 Differences in skin histological structure between high-altitude and low-altitude fishes. (A) The phylogeny, altitude and skin histological indicators (mean, standardized by the maximum) of experimental fishes. (B–G) The skin histological structure of three representative high-altitude fishes and their low-altitude relatives. The scale is 50 μm. RET, the relative epidermal thickness; RST, the relative scale thickness; RDT, the relative dermal thickness; MAMC, the mean area of mucus cells; MACC, the mean area of club cells; RTAMC, the relative total area of mucous cells; RTACC, the relative total area of club cells.
In Cobitoidea, the RDT, MAMC and RTAMC of the four high-altitude fishes (Triplophysa siluroides, Trilophysa bleekeri, Homatula potanini, and Beaufortia kweichowensis) were significantly lower than those of the low-altitude fishes. However, there were no uniform differences in other skin histological indicators between high-altitude fishes and low-altitude fishes. In addition, the scales of Triplophysa siluroides, Trilophysa bleekeri, and Homatula potanini were nearly lacking (Figure 2 and Table S2).
In Siluriformes, there were no significant differences in any skin histological indicators between the high-altitude species (Euchiloglanis kishinouyei) and its low-altitude relatives (Hemibagrus macropterus). However, the RET, RDT, MACC, and RTAMC of Euchiloglanis kishinouyei were significantly lower than those of another low-altitude fish (Silurus meridionalis) (Figure 2 and Table S2).
Interspecific comparisons of UVR damage
No mortality occurred in any control species. There were large differences in UVR tolerance among species, and the histological figures of each experimental fish before and after UVR exposure are shown in Figure S2. All individuals of Spinibarbus sinensis (4), Sinibrama taeniatus (9), Aristichthys nobilis (10) and Ictiobus Cyprinellus (20) died after 7 days of UVR exposure, and the skin of the dead individuals showed obvious damage (shedding and necrosis). All individuals of Beaufortia kweichowensis (19) and Silurus meridionalis (21) died after 7 days of UVR exposure, but the skin of the dead individuals showed no obvious damage, so these two species were excluded from subsequent analyses. A mean damage level less than 2 appeared 5 times independently in Schizothorax davidi (6), Gymnocypris eckloni (7), Rhynchocypris lagowskii and Tinca tinca (12-13), Triplophysa bleekeri and Triplophysa siluroides (17-18), and Oncorhynchus mykiss (22). Among them, Gymnocypris eckloni and Oncorhynchus mykiss showed the lowest damage levels (Figure 3 and Table S3). On the whole, the UVR damage level of high-altitude fishes was significantly lower than that of low-altitude fishes, and that of cold water fishes was significantly lower than that of eurythermal fishes (Table S4).
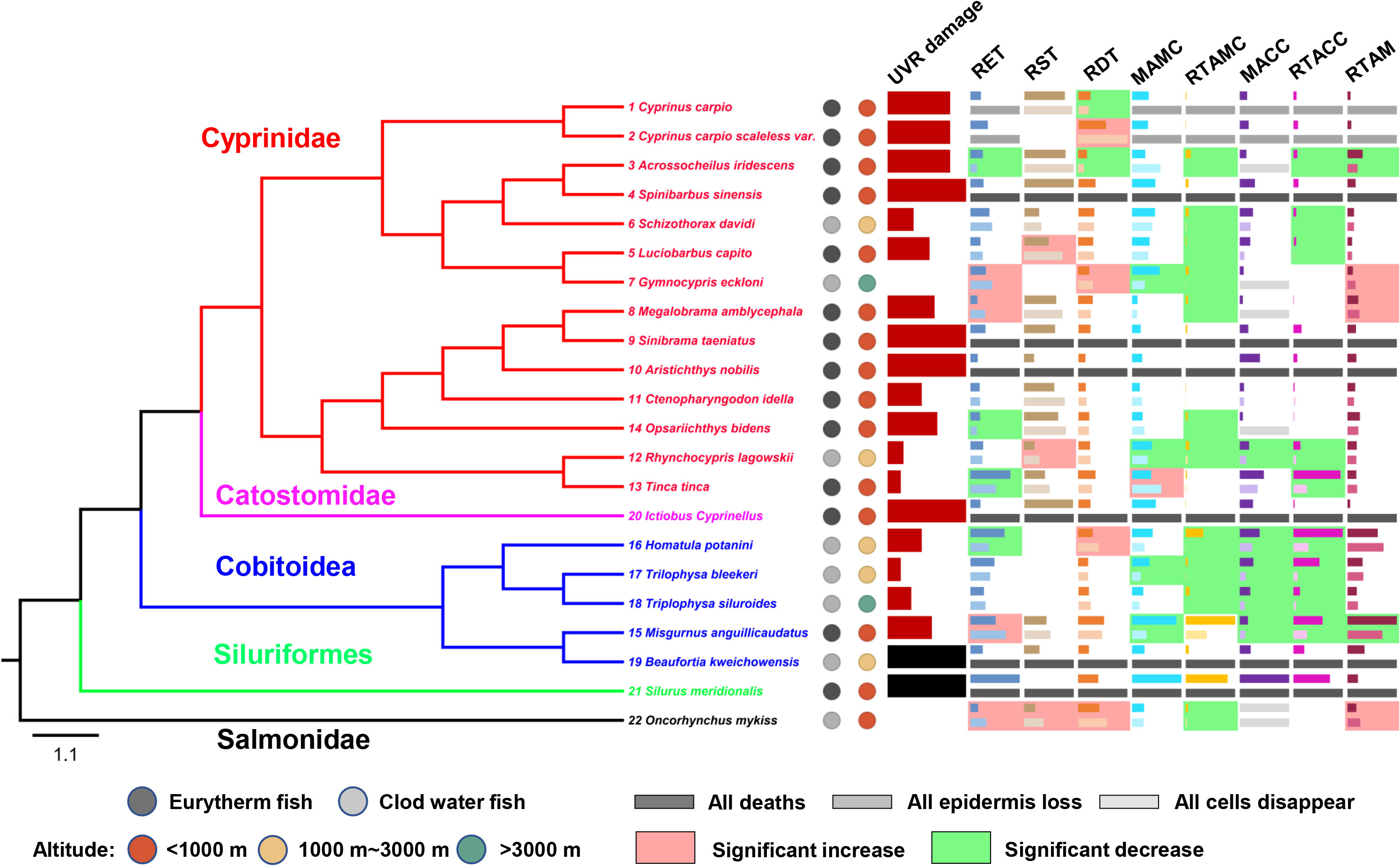
Figure 3 Interspecific differences in UVR damage and skin histological changes of experimental fishes. The black column for UVR damage indicates species that died but had no obvious skin damage and were thus excluded from subsequent analyses. For each skin histological indicator (mean, standardized by the maximum), the dark columns represent the values of the control groups, and the light columns represent the values of the experimental groups. The light red/green background represents a significant increase/decrease (P<0.05) after UVR exposure. The number before the Latin binomial is the serial number of the corresponding species. RET, the relative epidermal thickness; RST, the relative scale thickness; RDT, the relative dermal thickness; MAMC, the mean area of mucus cells; MACC, the mean area of club cells; RTAMC, the relative total area of mucous cells; RTACC, the relative total area of club cells.
Interspecific comparisons in skin histological changes after UVR exposure
The epidermis of Cyprinus carpio (1) and Cyprinus carpio scaleless var. (2) was completely lost, and that of Luciobarbus capito (5), Megalobrama amblycephala (8), Ctenopharyngodon idella (11) and Opsariichthys bidens (14) was partially lost. The RET of Acrossocheilus iridescens (3), Tinca tinca (13), Opsariichthys bidens (14), Homatula potanini (16) decreased significantly after UVR exposure, and that of Luciobarbus capito (5), Gymnocypris eckloni (7), Megalobrama amblycephala (8), Misgurnus anguillicaudatus (15), Oncorhynchus mykiss (22) increased significantly (Figure 3 and Table S5).
The RST of most species did not change significantly after UVR exposure. However, the RST of Luciobarbus capito (5), Rhynchocypris lagowskii (12) and Oncorhynchus mykiss (22) increased significantly after UVR exposure (Figure 3 and Table S5).
The RDT of most species did not change significantly after UVR exposure. However, the RDT of Cyprinus carpio (1) and Acrossocheilus iridescens (3) decreased significantly after UVR exposure, and that of Cyprinus carpio scaleless var. (2), Gymnocypris eckloni (7), Homatula potanini (16) and Oncorhynchus mykiss (22) increased significantly (Figure 3 and Table S5).
The mucous cells of Acrossocheilus iridescens (3), Luciobarbus capito (5), Megalobrama amblycephala (8) was completely disappeared, and the MAMC of Gymnocypris eckloni (7), Rhynchocypris lagowskii (12), Misgurnus anguillicaudatus (15) and Trilophysa bleekeri (17) decreased significantly after UVR exposure. However, the MAMC of Tinca tinca (13) increased significantly (Figure 3 and Table S5).
The club cells of Acrossocheilus iridescens (3), Luciobarbus capito (5), Schizothorax davidi (6) and Opsariichthys bidens (14) was completely disappeared, and the MACC of Rhynchocypris lagowskii (12), Misgurnus anguillicaudatus (15), Homatula potanini (16), Trilophysa bleekeri (17), Trilophysa siluroides (18) decreased significantly after UVR exposure (Figure 3 and Table S5).
The RTAMC and RTACC decreased in almost all species, and some fishes were significantly decrease, refer to Figure 3 and Table S5 for details.
The RTAM of Acrossocheilus iridescens (3) and Misgurnus anguillicaudatus (15) decreased significantly after UVR exposure, and that of Gymnocypris eckloni (7), Megalobrama amblycephala (8) and Oncorhynchus mykiss (22) increased significantly (Figure 3 and Table S5).
We did not find a significant difference in skin histological change rate between two groups after UVR exposure. The experimental fishes were divided into eurythermal and cold water groups, and we found the RTAM change rate of cold water group was increasing while that of eurythermal group was decreasing, and there was a significant difference between them (Table S6 and Figure S3).
We compared the correlation between the UVR damage and the change rate of skin structure before and after UVR exposure, and found that only the change rata of RTAM was strong negatively correlated (r=-0.76, P=0.002) with the level of damage (Table S7).
Correlation between UVR damage and skin structure
We examined the correlations between the UVR damage level and skin tissue structure parameters of the control group and found that only relative scale thickness was significantly positively correlated (r= 0.63, P=0.003 based on Pearson correlation analysis) with UVR damage level (Table S8, Figure 4A). In a previous study (Gu et al., 2022), we identified 4 factors that contribute to the increase in the number of fish scales (and the reduction in their thickness), namely, low-temperature adaptation, benthic adaptation, pelagic (upper-middle water column) adaptation and gene mutation [e.g., the fibroblast growth factor receptor 1 (Fgfr1) gene (Rohner et al., 2009)]. Interestingly, when we excluded the species with benthic adaptation, pelagic adaptation and mutation, the UVR damage level of the remaining species was strongly significantly positively correlated (r=0.92, P=3.6×10-6 based on Pearson correlation analysis; r=0.75, P=0.003 based on PIC correlation analysis) with the relative scale thickness (Figures 4B and 4C). The number of scales in these remaining species is mainly affected by temperature, with lower temperatures associated with more numerous (and thinner) scales in general (Gu et al., 2022), suggesting that low-temperature adaptation may be related to UVR adaptation. Indeed, the UVR damage in cold water fish was significantly lower than that in eurythermal fish (P=2.0×10-4) (Figure 5). Tinca tinca (13) was an exception. It is a eurythermal fish but is distributed at high latitudes (northern Eurasia) and shows strong adaptability to low temperature.
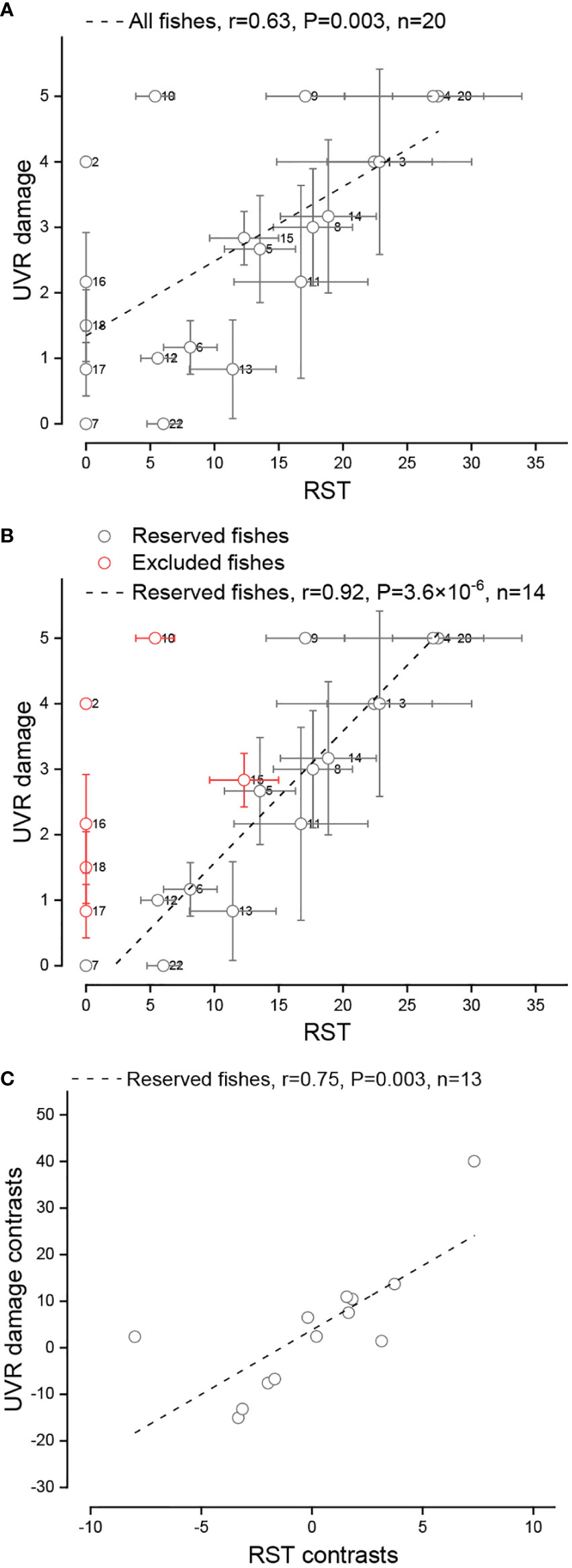
Figure 4 Relationship between the relative scale thickness (RST) and UVR damage of experimental fishes. (A) Traditional correlation between the RST and UVR damage in all fishes. (B) Traditional correlation between the RST and UVR damage in reserved fishes. (C) Correlation between the RST and UVR damage in reserved fishes as measured by PIC analysis. The circle and whiskers indicate the mean ± SD. A data point will be lost in PIC analysis.
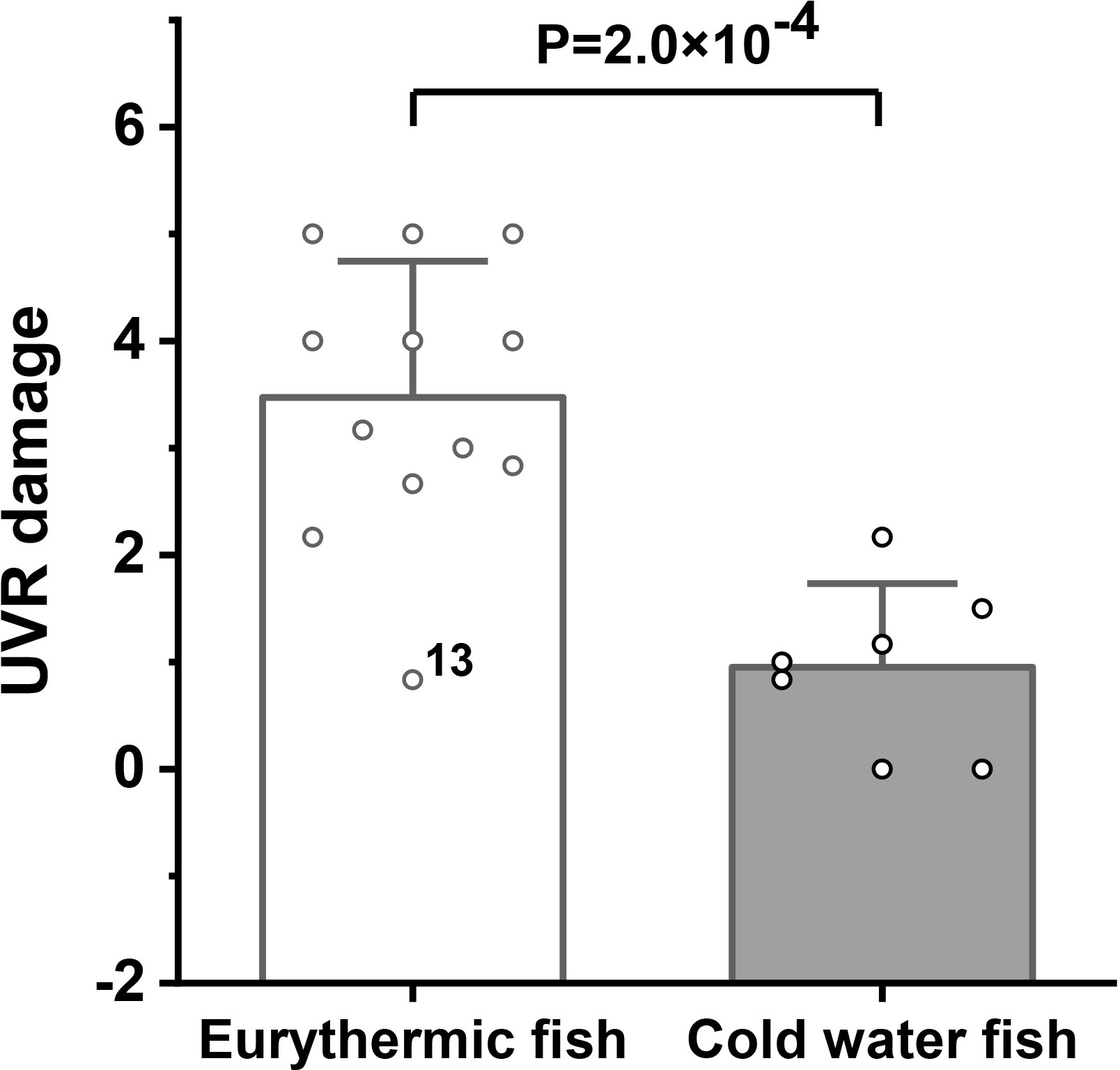
Figure 5 Comparison of UVR damage between eurythermal and cold water fishes. The height and whiskers indicate the mean ± SD.
Discussion
Skin histological differences between high-altitude and low-altitude fishes
High-altitude fishes all show small and thin scales or even the absence of scales. In Cyprinidae, with the uplift of the Tibetan Plateau, primitive large-scale Barbinae fish evolved into thin-scale or scaleless Schizothoracinae fish, which was mainly related to low-temperature adaptation (Cao et al., 1981; Gu et al., 2022). However, in Cobitoidea and Siluriformes, the scales were originally degenerated or lacking, and previous studies suggested that these fishes can escape UVR via a burrowing and benthic lifestyle (Cao et al., 1981). The lateral skin of G. eckloni had almost no club cells, similar to that of Oncorhynchus mykiss. Interestingly, among all the experimental fishes, these two species had the least skin damage after UVR exposure. The absence of club cells leads to an increased proportion of epithelial cells in the epidermis, indicating that epithelial cells may have played an important role in UVR resistance. In Cobitoidea, most high-altitude species lack a burrowing lifestyle, so they do not need to secrete much mucus, which may be why the size and total area of mucous cells in high-altitude species (mainly with a benthic lifestyle) were lower than those in low-altitude species (mainly with a burrowing lifestyle). In summary, thin scales or the absence of scales was common among high-altitude fishes in different taxa, but their other skin structure parameters did not display convergence.
Interspecific differences in skin histological changes after UVR exposure
Reduction in the number and size of fish mucous and rod cells after UVR exposure is common universal, and declining numbers of mucous cells in UVB-exposed fish might result from both a shorter cell half-life due to stimulated secretion and inhibition of cell regeneration (Kaweewat and Hofer, 1997). UVR exposure decreased the investment of experimental fish into club cells, and a previous study showed that short-term UVR exposure increased the physiological stress response in fishes, resulting in a reduction in epidermal club cell investment (Manek et al., 2012). However, the specific mechanism needs further study.
The epidermis is the carrier of mucous cells and club cells. After UVR exposure, the total area of mucous and club cells in fish decreased, which may have led to a decrease in their epidermal thickness. Indeed, the findings in some species support this hypothesis, such as Acrossocheilus iridescens and Tinca tinca. Interestingly, epidermal thickness in some species increased after UVR exposure, but the reasons for the thickening were different. In Gymnocypris eckloni and Oncorhynchus mykiss, the proliferation of epithelial cells led to epidermal thickening. In Megalobrama amblycephala, the vacuolation of epithelial cells led to epidermal thickening. In Misgurnus anguillicaudatus, the vacuolation of epithelial cells and an increased number of club cells led to epidermal thickening. The vacuolation of epithelial cells is a manifestation of UVR damage (Bullock, 1988; Ewing et al., 1999). Notably, the increase in epidermal cell investment may be an important reason for the strong UVR resistance of Gymnocypris eckloni and Oncorhynchus mykiss. Similarly, the dermal thickness significantly increased in Gymnocypris eckloni and Oncorhynchus mykiss. In addition, the change rata of melanin area was strong negatively correlated with the level of damage, where Gymnocypris eckloni and Oncorhynchus mykiss increased significantly, indicating that increased melanin was helpful to reduce UVR damage. In humans, exposure of skin to UVR leads to thickening of the epidermis and dermis, which along with pigmentation protects humans from UV-induced damage through reduced penetration of UV rays into the body (Pearse et al., 1987). Thickening is estimated to provide 10-fold higher protection from sunburn (McGregor and Hawk, 1999). In addition, epidermal thickening allows a larger number of cells, which increases the antioxidant potential of the epidermis (Lee et al., 2005). In summary, nonpathological skin thickening and melanin area increasing may reduce UVR damage, especially in UVR-resistant species.
Relationship between habitat altitude and temperature and its effect on fish UVR tolerance
Among all the skin indicators, only relative scale thickness had a positive correlation with UVR damage in the experimental fish. Two important factors affect the number or thickness of fish scales: temperature and water column position (Gu et al., 2022). Both benthic adaptation (such as in loaches and eels) and epipelagic adaptation (such as in silver carp) can lead the scales to become smaller and thinner (Gu et al., 2022). At colder temperatures, development (organ formation and differentiation) is slower than growth (individual size increase), and scales appear later when the individual is larger. Since the initial size of the scales is conservative, a larger individual will have more scales covering its body. Therefore, fish will have more scales under colder temperatures (Hubbs, 1922; Levin, 2010; Levin, 2011). Interestingly, when we excluded the species with benthic adaptation, pelagic adaptation and mutation, relative scale thickness showed a strong positive correlation with UVR damage in the experimental fish, indirectly indicating that UVR damage may be related to temperature adaptation.
Geographically, temperature is mainly affected by altitude and latitude; with increasing altitude, the stronger the UVR is, the lower the temperature. In addition, the upper reaches of rivers at higher altitudes are usually clear and shallow, providing weak UVR degradation, which may also lead to the long-term exposure of fish to high UVR. Therefore, long-term low-temperature and high-UVR adaptation may simultaneously affect UVR tolerance in fish. However, identifying the main driving factor is beyond the scope of the current study. Nevertheless, higher plants protect themselves against UV-induced damage by forming epidermally located phenolic compounds, such as flavonoids, which screen UVR (Emiliani et al., 2013). Interestingly, enhanced UVB radiation was probably not the key factor triggering shifts in the phenolic composition in flavonoids produced at higher altitudes but rather temperature, which decreases with altitude (Albert et al., 2009). Similarly, the acclimation of Arabidopsis thaliana to low temperature protects against UVR damage, and the main mechanism was enhanced epidermal screening, which reduces the epidermal transmittance of UVB irradiance (Schultze and Bilger, 2019). This evidence suggests that low-temperature adaptation plays an important role in UVR tolerance. Tinca tinca and Oncorhynchus mykiss possess small scales, and excellent low temperature adaptability. They are not high-altitude fish, but they possess high UVR tolerance, which also suggests the importance of low temperature adaptation for organismal UVR tolerance.
Data availability statement
The datasets presented in this study can be found in online repositories. The names of the repository/repositories and accession number(s) can be found in the article/Supplementary Material.
Ethics statement
All the animal procedures were conducted with the approval of the Committee of Laboratory Animal Experimentation at Southwest University, Chongqing, China and were in full compliance with the Committee's guidelines [protocol number (2014)25].
Author contributions
HG and ZW conceived the ideas and designed this study. HG wrote the original manuscript and completed data analysis. HG, SL, HW and SZ completed histology experiments. HG, ZW and DY participated in the discussion of the experimental results and the improvement of the manuscript. All authors contributed to the article and approved the submitted version.
Funding
This research was supported by the Fundamental Research Funds for the Central Universities (Grant No. SWU-KQ22016).
Acknowledgments
Thanks to all the authors for contributing to this article. Thanks to Jing Huang and Deyong Pu, two experimentalists of Key Laboratory of Freshwater Fish Reproduction and Development (Ministry of Education), for their help.
Conflict of interest
The authors declare that the research was conducted in the absence of any commercial or financial relationships that could be construed as a potential conflict of interest.
Publisher’s note
All claims expressed in this article are solely those of the authors and do not necessarily represent those of their affiliated organizations, or those of the publisher, the editors and the reviewers. Any product that may be evaluated in this article, or claim that may be made by its manufacturer, is not guaranteed or endorsed by the publisher.
Supplementary material
The Supplementary Material for this article can be found online at: https://www.frontiersin.org/articles/10.3389/fmars.2022.1035140/full#supplementary-material
References
Abedi S., Sharifpour I., Mozanzadeh M. T., Zorriehzahra J., Khodabandeh S., Gisbert E. (2015). A histological and ultrastructural study of the skin of rainbow trout (Oncorhynchus mykiss) alevins exposed to different levels of ultraviolet b radiation. J. Photochem. Photobiol. B. 147, 56–62. doi: 10.1016/j.jphotobiol.2015.02.021
Albert A., Sareedenchai V., Heller W., Seidlitz H. K., Zidorn C. (2009). Temperature is the key to altitudinal variation of phenolics in arnica montana l. cv. ARBO. Oecologia. 160, 1–8. doi: 10.1007/s00442-009-1277-1
Alves R. N., Mahamed A. H., Aiarcon J. F., Al Suwailem A., Agustin S. (2020). Adverse effects of ultraviolet radiation on growth, behavior, skin condition, physiology, and immune function in gilthead seabream (Sparus aurata). Front. Mar. Sci. 7. doi: 10.3389/fmars.2020.00306
Bae J., Kwon H., Park S. R., Lee J., Song I. (2006). Explicit correlation coefficients among random variables, ranks, and magnitude ranks. IEEE T Inform Theory. 52, 2233–2240. doi: 10.1109/TIT.2006.872852
Ballare C. L., Caldwell M. M., Flint S. D., Robinson S. A., Bornman J. F. (2011). Effects of solar ultraviolet radiation on terrestrial ecosystems. Patterns mechanisms Interact. Climate change. Photoch Photobio Sci. 10, 226–241. doi: 10.1039/c0pp90035d
Beckmann M., Vaclavik T., Manceur A. M., Sprtova L., von Wehrden H., Welk E., et al. (2014). glUV: a global UV-b radiation data set for macroecological studies. Methods Ecol. Evol. 5, 372–383. doi: 10.1111/2041-210X.12168
Blazer V. S., Fabacher D. L., Little E. E., Ewing M. S., Kocan K. M. (1997). Effects of ultraviolet-b radiation on fish: Histologic comparison of a UVB-sensitive and a UVB-tolerant species. J. Aquat Anim. Health 9, 132–143. doi: 10.1577/1548-8667(1997)009<0132:EOUBRO>2.3.CO;2
Bullock A. M. (1982). The pathological effects of ultraviolet-radiation on the epidermis of teleost fish with reference to the solar-radiation effect in higher animals. P Roy Soc. B-biol Sci. 81, 199–210. doi: 10.1017/S0269727000003390
Bullock A. M. (1988). Solar ultraviolet radiation: A potential environmental hazard in the cultivation of farmed finfish (Dordrecht: Springer).
Cao W. X., Chen Y. Y., Wu Y. F., Zhu S. Q. (1981). “Origin and evolution of schizothoracine fishes in relation to the upheaval of the xizang plateau,” in Studies on the period, ampli-tude and type of the uplift of the qinghai-xizang plateau (Beijing: Science Press).
Chivers D. P., Wisenden B. D., Hindman C. J., Michalak T., Kusch R. C., Kaminskyj S. W., et al. (2007). Epidermal 'alarm substance' cells of fishes maintained by non-alarm functions: possible defence against pathogens, parasites and UVB radiation. P Roy Soc. B-biol Sci. 274, 2611–2619. doi: 10.1098/rspb.2007.0709
Emiliani J., Grotewold E., Falcone F. M., Casati P. (2013). Flavonols protect arabidopsis plants against UV-b deleterious effects. Mol. Plant 6, 1376–1379. doi: 10.1093/mp/sst021
Ewing M. S., Blazer V. S., Fabacher D. L., Little E. E., Kocan K. M. (1999). Channel catfish response to ultraviolet-b radiation. J. Aquat Anim. Health 11, 192–197. doi: 10.1577/1548-8667(1999)011<0192:CCRTUB>2.0.CO;2
Fabacher D. L., Little E. E. (1995). Skin component may protect fishes from ultraviolet-b radiation. Environ. Sci. pollut. R. 2, 30–32. doi: 10.1007/BF02987508
Felsenstein J. (1985). Phylogenies and the comparative method. Am. Nat. 125, 1–15. doi: 10.1086/284325
Fukunishi Y., Masuda R., Yamashita Y. (2006). Ontogeny of tolerance to and avoidance of ultraviolet radiation in red sea bream pagrus major and black sea bream acanthopagrus schlegeli. Fisheries Sci. 72, 356–363. doi: 10.1111/j.1444-2906.2006.01157.x
Gautam S., Singh J., Pant A. B., Singh S. (2010). Response and adaptive strategies of Antarctic flora to ultraviolet radiation stress. J. Photoch Photobio C. 11, 247–247. doi: 10.1016/j.jphotochemrev.2010.07.001
Guo Y. S., Sun Z. Y., He X. H., Jin W., Chen Y. L. (2021). Colored atlas of fishes in sichuan (Beijing: Science Press).
Gu H. R., Wang H. Y., Zhu S. D., Yuan D. Y., Dai X. Y., Wang Z. J. (2022). Interspecific differences and ecological correlations between scale number and skin structure in freshwater fishes. Curr. Zool. XX, 1–10. doi: 10.1093/cz/zoac059
Holzle E., Honigsmann H. (2005). UV-Radiation-sources, wavelength, environment. J. Dtsch Dermatol. Ges. 3 Suppl 2, S3–10. doi: 10.1111/j.1610-0387.2005.04392.x
Hubbs C. L. (1922). Variations in the number of vertebrae and other meristic characters of fishes correlated with the temperature of water during development. Am. Nat. 56, 360–372. doi: 10.1086/279875
Hugie D. M. (1990). An intraspecific approach to the evolution of chemical alarm signalling in the ostariophysi. [disertation/master’s thesis] (Saskatoon: University of Saskatchewan).
Katoh K., Rozewicki J., Yamada K. D. (2019). MAFFT online service: multiple sequence alignment, interactive sequence choice and visualization. Brief Bioinform. 20, 1160–1166. doi: 10.1093/bib/bbx108
Kaweewat K., Hofer R. (1997). Effect of UV-b radiation on goblet cells in the skin of different fish species. J. Photoch Photobio B. 41, 222–226. doi: 10.1016/S1011-1344(97)00104-8
Kessler K., Lockwood R. S., Williamson C. E., Saros J. E. (2008). Vertical distribution of zooplankton in subalpine and alpine lakes: Ultraviolet radiation, fish predation, and the transparency-gradient hypothesis. Limnol Oceanogr. 53, 2374–2382. doi: 10.4319/lo.2008.53.6.2374
Leech D. M., Boeing W. J., Cooke S. L., Williamson C. E., Torres L. (2009). UV-Enhanced fish predation and the differential migration of zooplankton in response to UV radiation and fish. Limnol Oceanogr. 54, 1152–1161. doi: 10.4319/lo.2009.54.4.1152
Lee D. S., Quan G., Choi J. Y., Kim S. Y., Lee S. C. (2005). Chronic ultraviolet radiation modulates epidermal differentiation as it up-regulates transglutaminase 1 and its substrates. Photodermatol Photo. 21, 45–52. doi: 10.1111/j.1600-0781.2005.00131.x
Levin B. A. (2010). Drastic shift in the number of lateral line scales in the common roach rutilus rutilus as a result of heterochronies: experimental data. J. Appl. Ichthyol. 26, 303–306. doi: 10.1111/j.1439-0426.2010.01426.x
Levin B. A. (2011). Ontogenetic causes and mechanisms for formation of differences in number of fish scales. Russ J. Dev. Biol. 42, 186–191. doi: 10.1134/S1062360411030106
Liu K., Yang J., Yuan H. (2021a). Recent progress in research on the gut microbiota and highland adaptation on the qinghai-Tibet plateau. J. Evol. Biol. 34, 1514–1530. doi: 10.1111/jeb.13924
Liu C., Zhu L. P., Li J. S., Wang J. B., Ju J. T., Qiao B. J., et al. (2021b). The increasing water clarity of Tibetan lakes over last 20 years according to MODIS data. Remote Sens Environ. 253, 112199. doi: 10.1016/j.rse.2020.112199
Manek A. K., Ferrari M. C., Chivers D. P., Niyogi S. (2014). Dissolved organic carbon ameliorates the effects of UV radiation on a freshwater fish. Sci. Total Environ. 490, 941–946. doi: 10.1016/j.scitotenv.2014.05.102
Manek A. K., Ferrari M. C. O., Sereda J. M., Niyogi S., Chivers D. P. (2012). The effects of ultraviolet radiation on a freshwater prey fish: physiological stress response, club cell investment, and alarm cue production. Biol. J. Linn Soc 105, 832–841. doi: 10.1111/j.1095-8312.2011.01829.x
Mao K., Wang Y., Liu J. (2021). Evolutionary origin of species diversity on the qinghai-Tibet plateau. J. Syst. Evol. 59, 1142–1158. doi: 10.1111/jse.12809
McFadzen I., Baynes S., Hallam J., Beesley A., Lowe D. (2000). Histopathology of the skin of UV-b irradiated sole (Solea solea) and turbot (Scophthalmus maximus) larvae. Mar. Environ. Res. 50, 273–277. doi: 10.1016/S0141-1136(00)00085-4
McGregor J. M., Hawk J. L. M. (1999). Dermatology ingeneral medicine. Eds. Freedberg I. M., Eisen A. Z., Wolff K., Austen K. F., Goldsmith L. A., Katz S. I. (New York: McGraw Hill). Dermatology ingeneral medicine.
Norsang G., Jin Y. M., Tsoja W. M., Zhou Y., Sunam B., Tunzhu D. (2019). Ground-based measurements of global solar radiation and UV radition in Tibet. Spectrosc Spect Anal. (in Chinese). 39, 1683–1688. doi: 10.3964/j.issn.1000-0593(2019)06-1683-06
Paradis E., Claude J., Strimmer K. (2004). APE: Analyses of Phylogenetics and Evolution in R language. Bioinformatics 20, 289–290. doi: 10.1093/bioinformatics/btg412
Paul N. D., Gwynn-Jones D. (2003). Ecological roles of solar UV radiation: towards an integrated approach. Trends Ecol. Evol. 18, 48–55. doi: 10.1016/S0169-5347(02)00014-9
Pearse A. D., Gaskell S. A., Marks R. (1987). Epidermal changes in human skin following irradiation with either UVB or UVA. J. Invest. Dermatol. 88, 83–87. doi: 10.1111/1523-1747.ep12465094
Peng S. M., Du Q. Y., Wang C. L., Lin W. A., Hu B. (2015). Long-term variations of ultraviolet radiation in Tibetan plateau from observation and estimation. Int. J. Climatol. 35, 1245–1253. doi: 10.1002/joc.4051
Qu Y., Zhao H., Han N., Zhou G., Song G. (2013). Ground tit genome reveals avian adaptation to living at high altitudes in the Tibetan plateau. Nat. Commun. 4, 2071. doi: 10.1038/ncomms3071
Rohner N., Bercsenyi M., Orban L., Kolanczyk M. E., Linke D., Brand M., et al. (2009). Duplication of fgfr1 permits fgf signaling to serve as a target for selection during domestication. Curr. Biol. 19, 1642–1647. doi: 10.1016/j.cub.2009.07.065
Schultze M., Bilger W. (2019). Acclimation of arabidopsis thaliana to low temperature protects against damage of photosystem II caused by exposure to UV-b radiation at 9 degrees c. Plant Physiol. Biochem. 134, 73–80. doi: 10.1016/j.plaphy.2018.10.017
Sucre E., Vidussi F., Mostajir B., Charmantier G., Lorin-Nebel C. (2012). Impact of ultraviolet-b radiation on planktonic fish larvae: alteration of the osmoregulatory function. Aquat Toxicol. 109, 194–201. doi: 10.1016/j.aquatox.2011.09.020
Trifinopoulos J., Nguyen L. T., von Haeseler A., Minh B. Q. (2016). W-IQ-TREE: a fast online phylogenetic tool for maximum likelihood analysis. Nucleic Acids Res. 44, W232–W235. doi: 10.1093/nar/gkw256
Wang X., Wang Y., Li Q., Tseng Z. J., Takeuchi G. T., Deng T., et al. (2015). Cenozoic Vertebrate evolution and paleoenvironment in Tibetan plateau: Progress and prospects. Gondwana Res. 27, 1335–1354. doi: 10.1016/j.gr.2014.10.014
Wang M., Yang J. X., Chen X. Y. (2013). Molecular phylogeny and biogeography of percocypris (Cyprinidae, teleostei). PLoS One 8, e61827. doi: 10.1371/journal.pone.0061827
Weise Z., Nuo S., Jin Y. M., Cuojia W., Nuo Z. (2018). Study on solar UV radiation measurement of mount Everest in Tibet. Energy Energy Conserv. 04, 60–62. doi: 10.16643/j.cnki.14-1360/td.2018.04.028
Williamson C. E., Neale P. J., Hylander S., Rose K. C., Figueroa F. L., Robinson S. A., et al. (2019). The interactive effects of stratospheric ozone depletion, UV radiation, and climate change on aquatic ecosystems. Photochem. Photobiol. Sci. 18, 717–746. doi: 10.1039/c8pp90062k
Xiao S., Mou Z., Fan D., Zhou H., Zou M., Zou Y., et al. (2020). Genome of tetraploid fish schizothorax oconnori provides insights into early re-diploidization and high-altitude adaptation. Iscience. 23, 101497. doi: 10.1016/j.isci.2020.101497
Yang T., Liang W. Y., Cai J. H., Gu H. R., Han L., Chen H. Y., et al. (2021a). A new cyprinid from the oligocene of qaidam basin, north-eastern Tibetan plateau, and its implications. J. Syst. Palaeontol. 19, 1161–1182. doi: 10.1080/14772019.2021.2015470
Yang L., Sado T., Vincent H. M., Pasco-Viel E., Arunachalam M., Li J. B., et al. (2015). Phylogeny and polyploidy: resolving the classification of cyprinine fishes (Teleostei: Cypriniformes). Mol. Phylogenet Evol. 85, 97–116. doi: 10.1016/j.ympev.2015.01.014
Yang L., Wang Y., Sun N., Chen J., He S. (2021b). Genomic and functional evidence reveals convergent evolution in fishes on the Tibetan plateau. Mol. Ecol. 30, 5752–5764. doi: 10.1111/mec.16171
Yuan D. Y., Chen X. H., Gu H. R., Zou M., Zou Y., Fang J., et al. (2020). Chromosomal genome of triplophysa bleekeri provides insights into its evolution and environmental adaptation. Gigascience. 9, giaa132. doi: 10.1093/gigascience/giaa132
Zagarese H. E., Williamson C. E. (2001). The implications of solar UV radiation exposure for fish and fisheries. Fish Fish. 2, 250–260. doi: 10.1046/j.1467-2960.2001.00048.x
Zhang D., Gao F. L., Jakovlic I., Zou H., Zhang J., Li W. X., et al. (2020). PhyloSuite: An integrated and scalable desktop platform for streamlined molecular sequence data management and evolutionary phylogenetics studies. Mol. Ecol. Resour. 20, 348–355. doi: 10.1111/1755-0998.13096
Keywords: skin structure, histology, UVR damage, low-temperature adaptation, plateau fish, high-altitude adaptation
Citation: Gu H, Li S, Wang H, Zhu S, Yuan D and Wang Z (2022) Interspecific differences and ecological correlations of ultraviolet radiation tolerance in low- and high-altitude fishes. Front. Mar. Sci. 9:1035140. doi: 10.3389/fmars.2022.1035140
Received: 02 September 2022; Accepted: 24 October 2022;
Published: 09 November 2022.
Edited by:
José Ricardo Paula, MARE - Marine and Environmental Sciences Centre, PortugalReviewed by:
Ricardo Nuno Alves, King Abdullah University of Science and Technology, Saudi ArabiaChristina Pasparakis, University of California, Davis, United States
Marta Monteiro, University of Aveiro, Portugal
Copyright © 2022 Gu, Li, Wang, Zhu, Yuan and Wang. This is an open-access article distributed under the terms of the Creative Commons Attribution License (CC BY). The use, distribution or reproduction in other forums is permitted, provided the original author(s) and the copyright owner(s) are credited and that the original publication in this journal is cited, in accordance with accepted academic practice. No use, distribution or reproduction is permitted which does not comply with these terms.
*Correspondence: Zhijian Wang, wangzj1969@126.com